
Data are obtained by orienting panels face to face with the separation equal to twice the spacer thickness. In addition, we find the metastable states of green, blue, and red panels. The globally optimal ground state occurs when the panels are face to face with no separation.
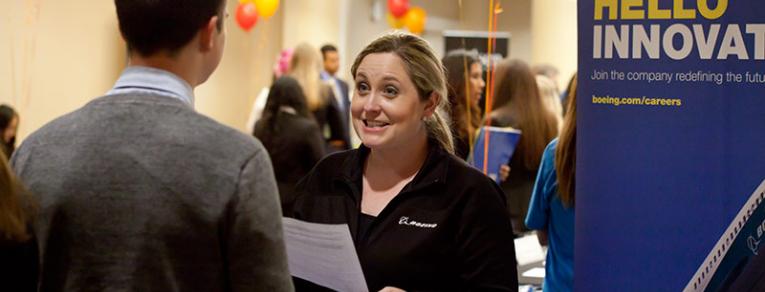

( F) Theoretical calculation of the binding-energy landscape of 2 panels of the same dipole pattern as a function of the lateral separation normalized by the dipole separation within a panel. ( E) Theoretical calculation of the ground-state binding energy between any 2 panels. ( D) Schematic illustrating the interactions between panels of different orientations (top left) and dipole patterns (bottom right). Data are obtained by placing panels face to face, releasing them and determining if they spontaneously bind. ( C) Plot of the range for spontaneous binding (normalized by the dipole separation within a panel) versus Γ, the shaking acceleration normalized by g. These spacers create a steric repulsion at a surface separation corresponding to the vertical dashed line. We glue spacers onto the magnetically patterned layers to further enhance the selectivity of the panel interactions. Solid lines are experimental measurements, and dashed lines are results from analytic calculations in which interactions between any 2 pairs of magnets that are not in the same magnetically patterned layer are considered. Here, we normalize the separation by the lateral dipole separation within a magnetically patterned layer. ( B) Magnetic force between 2 magnetically patterned layers of the same pattern at the optimal binding orientation (sketch in Inset) versus the center-to-center layer separation. Spacers are glued to the 2 faces of the magnetically patterned layer to make a panel. The magnetically patterned layers are arbitrarily colored black, green, blue, and red according to their dipole patterns. ( A) Four unique magnetically patterned layers are constructed by using a 2 × 2 array of magnets with different orientations (top and side views). Encoding information into magnetic panels to generate specific interactions. To develop complementary platforms that overcome such challenges at the microscale and nanoscale, it is necessary to develop strategies based on building blocks that encode binding interactions that are both stronger and longer-ranged.įig. Moreover, the resulting materials are inherently soft since thermal fluctuations are necessary for these systems to explore their phase space and arrive at optimal binding configurations. Despite tremendous successes achieved by these pioneering studies, these binding strategies typically only function at small size scales and often suffer from long annealing times, as elements must diffuse into near contact to interact ( 20, 21). These latter strategies have been successful at generating complex structures ranging from clathrate colloidal crystals ( 19) to complex polyhedra and nanoscale DNA teddy bears ( 14). Other pioneering studies incorporated enthalpic interactions through DNA-mediated colloidal ( 2, 6– 12) and DNA origami/Lego self-assembly platforms ( 13– 18). For example, previous work has shown that entropic bonds arising from particle shape alone can lead to formation of complex crystal structures ( 3– 5).

At the microscale and nanoscale, such rules have been encoded through entropic and enthalpic interactions ( 1– 3). Programmable self-assembly of complex arrangements from simple units requires encoding local interaction rules into building blocks to select a desired final structure. We envision that magnetic encoding of assembly instructions into primary structures of panels, strands, and nets will lead to the formation of secondary and even tertiary structures that transmit information, act as mechanical elements, or function as machines on scales ranging from the nano to the macro. Using a centimeter-sized version of these panels, we demonstrate 3 canonical hallmarks of assembly: controlled polymerization of individual building blocks assembly of 1-dimensional strands made of panels connected by elastic backbones into secondary structures and hierarchical assembly of 2-dimensional nets into 3-dimensional objects. Because the ratios of the different panel-binding energies are scale-invariant, this approach can, in principle, be applied down to the nanometer scale. Our building blocks consist of panels with different patterns of magnetic dipoles that are capable of specific binding. Here, we introduce a platform based on magnetic encoding of information to drive programmable self-assembly that works across length scales. Programmable self-assembly of smart, digital, and structurally complex materials from simple components at size scales from the macro to the nano remains a long-standing goal of material science.
